Geothermal energy can bridge the transition away from fossil fuels, as it currently makes use of the tools and existing wells in the oil and gas industry, while also addressing the unreliability of current renewables.
Current Developments, Issues, Discoveries and Advancements in Geothermal Energy
Dr. Raj Shah Ms. Cindy Huang, Mr.Gavin Thomas Mr.Nikhil Pai Ms.Mrinaleni Das Mr. Nicholas Douglas | Koehler Instrument Company
The past few years have seen increasing interest in renewable energy due to greater awareness of climate change and sustainability. Geothermal energy is one of several potentially viable replacements for the modern fossil fuel industry. Fossil fuels exploit the chemical energy found in the remains of once-living organisms through combustion. These resources, in the form of crude oil, natural gas, and coal, are non-renewable and release pollutants such as carbon dioxide and nitrous oxide. In contrast, geothermal energy is semi-renewable [1], with potential to become renewable as technology improves, and emits no greenhouse gases [2]. Geothermal energy utilizes heat from the slow decay of radioactive particles below the Earth’s surface, extracted in the form of hot water and steam [2, 3].
One of the unique advantages of geothermal energy compared to other renewable sources is its ability to be utilized at all hours of the day and in any weather condition. Other alternative forms of renewable energy–hydropower, solar energy, and wind power–are variable sources [4] that are dependent on environmental conditions and fluctuate in power generation. This dependency poses a restriction for the applicability of the variable sources and thus hinders their prospect in fully replacing the fossil fuel industry.
Geothermal energy can bridge the transition away from fossil fuels, as it currently makes use of the tools and existing wells in the oil and gas industry, while also addressing the unreliability of current renewables. The variable aspect of hydropower, solar, and wind power means production may not keep up during peak consumption hours, but geothermal systems can supplement this variability or serve as batteries to store excess energy produced during low demand hours. However, geothermal energy is still in the fledgling stages, existing only in small corners of the world. If geothermal energy is to be implemented worldwide as a primary energy source, more focus needs to be directed towards the research and development of new technologies to enable its global utilization. This work intends to give an overview of current geothermal heating and electricity systems and explore new innovations in enhanced and advanced geothermal systems being developed globally to increase viability worldwide.
Current State of Geothermal Heating
Most of the harvested geothermal is used directly for heating and cooling systems [1]. Geothermal systems have the advantage of being able to provide direct heating and cooling, thereby reducing the burden on other renewable sources. Hydro, solar, and wind power can only generate electricity which must be converted to thermal energy for space heating and cooling. In buildings with electric powered space conditioning systems, approximately 40-50% of electricity consumption is due to heating and cooling [5].
In addition, geothermal heating and cooling systems require much less strict temperature requirements for utilization and can operate at medium (90-150℃) and low (<90℃) geothermal temperatures [4]. Low and medium-temperature sites are more widely available and can be found concentrated along faults and fractures in tectonically active areas and in sedimentary basins [4]. The wider range of implementation means geothermal heating systems currently have more installed power capacity than geothermal electricity plants and can exist in a wider range of locations (Figure 1).
Lower temperatures are suitable for a wide range of applications in industries such as manufacturing, district heating, and agriculture. Some examples of processes where geothermal heat may be applied include pasteurization, distillation, extraction, and sterilization [4].
Geothermal heat pumps are a small-scale form of application that makes use of extremely low geothermal temperatures for individual buildings. Only a few feet below the surface, the ground remains at constant temperatures of 7-21°C. These constant temperatures are used for cooling in the summer and warming in the winter. These heat pumps use closed-loop pipes containing antifreeze fluid placed below the surface [6].
On a larger scale, district heating systems have also advanced to allow large heat pumps to work at temperatures less than 50℃ [4]. However, these require buildings that are well-insulated to prevent heat loss.
Figure 1. Geothermal heating and cooling installed capacity globally in 2020 [4].
Current State of Electricity Generation
In 2020, geothermal electricity made up less than 1% or 14 GW (Figure 2), of the globally installed electricity capacity (7735 GW); comparatively, fossil fuels made up 60%, or 4433 GW [7]. The United States has the greatest capacity for electricity generation, with 20% of global generation at 3700 MW [7, 8]. Despite this, geothermal electricity also constitutes less than 1% of the country's electricity generation [1].
Figure 2. Globally installed geothermal electricity capacity in 2021 [4].
Geothermal energy can be accessed anywhere throughout the world but is heavily underutilized (Figure 3). This is due to the need for highly specific site conditions at commercial levels: temperatures greater than 150℃, a permeable substrate, and an existing self-replenishing water or steam reservoir (Figure 4) [4]. These conditions are primarily found in tectonically or volcanically active regions, as shown by the red zones in Figure 2. As a result, most geothermal power plants are concentrated in small corners of the world (Figure 2) [4].
Figure 3. Global map showing electricity capacity at depths where temperature is over 150℃ [9].
Figure 4. Diagram of Ideal Geothermal Site [5].
The technology for electricity generation under high temperature and permeability conditions is well developed, with commercial electricity generation in place since 1913 [10]. There are three main methods of converting geothermal resources to electricity: dry steam, flash steam, and binary cycles.
Dry steam plants (Figure 5) make use of steam extracted directly from the reservoir at high pressure to power steam turbines [4]. Condensed steam can then be discharged directly into the atmosphere or back into the reservoir [11].
In flash steam plants (Figure 5), geothermal fluids are extracted under high pressure and then transported into a low-pressure chamber at the surface. The vaporization process at low pressure is a process known as “flashing” [11]. The steam is used to power steam turbines, while the remaining liquid can be flashed again for more steam. Any remaining fluid is reinjected into the reservoir, like with dry steam processes.
As for binary-cycle power plants—like those in Figure 5—they are like dry steam and flash plants, except for the geothermal fluid not being directly used to power the steam turbines. Heat is transferred to a second working fluid in a Rankine cycle (butane or pentane) or a Kalina cycle (ammonia and water) [11]. With this process, all the geothermal fluid can be reinjected back into the reservoir, as none is lost as steam. Binary-cycle plants have the capability of using geothermal fluids at lower temperatures than dry steam and flash steam plants [11], thereby increasing viability in more locations globally. Currently, in small-scale applications, temperatures as low as 70-80℃ can be used to generate electricity [4].
Figure 5. Schematics of the three main forms of geothermal power plants [4].
Enhanced Geothermal Systems
One of the conditions needed for hydrothermal systems, in addition to high temperatures at a shallow depth, is a suitable reservoir. Qualifying geothermal reservoirs are regions below the surface that are porous and highly permeable to allow fluid movement through the injection and the productions wells [12]. In locations without porous and permeable substrate but has high temperatures at shallow depths, artificial reservoirs can be constructed by drilling two wells and inducing secondary porosity and permeability to connect the two (Figure 6) [12, 13]. A circulating fluid can then be injected from one well through the man-made fissures, absorbing heat before emerging out the other well [4]. These artificial reservoirs are called enhanced geothermal systems, also known as human-made geothermal energy.
Figure 6. Schematic of an enhanced geothermal process [13].
To successfully make the transition away from oil, new drilling equipment will have to be developed for geothermal applications. The problem with existing oil and gas is that they are not meant to withstand temperatures greater than 170-180°C [14]. However, for geothermal use, these temperatures just pass the minimum temperature needed to generate electricity.
Fervo, a start-up, currently operates enhanced geothermal plants in Utah and Nevada, two states historically known for having abundances of shallow geothermal resources [15]. However, due to years of depletion in shallow hot basins found in Utah and Nevada, deeper wells will need to be dug in future years to reach untapped geothermal resources [14] (Figure 7). Currently, the oil and gas technology only allow for drilling up to 4000 meters in the Western United States. At depths of 4500 meters and lower, temperatures reach approximately 175-200°C; drilling deeper than 5500 meters would reach temperatures closer to 200℃ (Figure 8) [16]—unsuitable for current drilling tools.
Figure 7. Displays relative favorability for potential deep enhanced geothermal systems based on the availability of regions with temperatures greater than 150°C, from 3-10 km below the surface [17].
Figure 8. Temperatures below the surface at 4.5 and 5.5 km [16].
In addition to higher temperatures, drilling equipment needs to be adapted for tougher materials. Tests done at The Geysers, a geothermal site composed of metasandstone, a metamorphic rock, showed the drill bit travelling only approximately three meters per hour and lasted for only thirty meters [18]. Drills from the oil and gas industry were intended for sedimentary rocks such as shale, mudstone, and limestone [19], which are softer than igneous and metamorphic rocks [12]. Existing traditional geothermal wells also primarily exist in sedimentary hot basins [15], due to the greater porosity of sedimentary rocks [12], making them the only substrate containing the fluids needed for traditional geothermal systems. However, enhanced geothermal sites are primarily located in granite substrate (Figure 10) [20]. Granite has low primary porosity [12], making it a poor location for traditional geothermal plants, but has good secondary porosity from fractures created post-rock formation [12], making the substrate a prime target for hydraulic fracturing.
Currently, the technology being developed to create artificial reservoirs is derived from hydraulic fracturing or “fracking,” which is primarily used by the oil and gas industry [15]. Fracking is the process of injecting an aqueous mixture of chemicals at high pressure into an existing well to induce fractures (Figure 9) [21]. These fractures allow the natural resources located within the substrate to flow out towards the surface for energy usage. However, unlike the oil and gas industry, no toxic chemicals are used for the geothermal adaptation of fracking - with water or carbon dioxide being used instead [20].
Figure 9. Diagram depicting the process of hydraulic fracturing [21].
Fenton Hill—the first enhanced geothermal site—proved to many that drilling deep into granite was possible [20], enhancing the development of fracking. Although Fenton Hill proved this possible [20], very few geothermal wells have since been drilled into tougher crystalline rocks [20, 22]. The significant lack of prior data means that there is difficulty in predicting the success rate, leading to the failures seen in all enhanced geothermal sites prior to Soultz [20].
Figure 10. Graph of reservoir lithology frequency for all existing enhanced geothermal sites. Mixed substrate refers to the site having a mixture of various sedimentary substrate in combination with igneous or metamorphic rocks. Adapted from data in [20].
One of the major difficulties with fracking is the inability to control or predict fractures. To create an efficient reservoir, fractures must be in the right orientation and be localized enough to prevent loss of fluid and heat [20]. The first few enhanced geothermal sites–Fenton Hill, Rosemanowes, Hijiori, and Ogachi–had all faced the same issues of circulating fluid loss (50-80%) due to thermal short circuits [20]. Hydraulic fractures are difficult to predict due to the complexity of subsurface conditions. Researchers have discovered that drilling into trans-tensional substrate is more likely to result in success as it prevents the fractures from sealing compared to compressional substrate [20], but little else is predictable. If high-temperature scanners are developed, more data can be collected to make more accurate predictions before drilling.
Primarily, fracking processes are used to induce earthquakes and create fractures. The pressure of injection can cause parts of the earth’s crust to crash or slide against each other, which creates earthquakes [23]. In Basel, Switzerland, during 2006, a few hydraulic fracturing tests conducted in granite at the north end of the city caused thousands of small earthquakes at the site and in the city of Basel. One of earthquake felt in the city had a magnitude of 3.4 and caused damage to multiple buildings [20]. This was not an isolated case either; in 2017 Pohang, South Korea, a 5.5-magnitude earthquake occurred at the injection site, and investigations revealed it was a result of hydraulic fracturing [4].
At The Geysers, a cold fracturing method was successfully tested and shown to reduce vibrations caused by fracking. This is due to the field abandoning the use of pressure pumps. Instead opting for stark temperature differences when using water at 13℃ to create fractures [20]. The highest earthquake caused by cold fracturing at The Geysers had a magnitude of 2.87 [20].
Supercritical Geothermal Sites
An optimistic goal some researchers have is to drill down past ten kilometers where temperatures average 400℃ (Figure 10) [14, 16] and remain in a supercritical state. Supercritical fluids have temperatures and pressures greater than 374°C and 221 bars [4] and can hold five to ten times more energy than normal steam [14]. The Iceland Deep Drilling Project, a project intent on developing methods to exploit reservoirs in a supercritical state, researchers estimated a borehole in Krafla has at least 36 MW of electricity production potential [24]; compared to a typical geothermal well that has an estimated potential of 5 MW [25], this is a significant difference.
Figure 11. Geothermal temperatures at 10 km [16].
Supercritical reservoirs are found deep in volcanic hydrothermal systems in countries such as Iceland, Japan, Kenya, Mexico, and New Zealand (Figure 12) [4]. The technology needed for drilling at such temperatures and pressures is currently possible but inefficient. The issues faced are the same as in drilling enhanced geothermal systems, with the addition of the corrosive nature of supercritical fluids [18, 25]. Currently, developments are being made to improve drilling efficiency and scalability. GA Drilling, a company based in Slovakia, is working on plasma tools to withstand these conditions [14]. Quaise, a Massachusetts start-up, is also in the development of millimeter wave tools that could potentially pulverize rocks and reach depths of over 20 kilometers [14].
Figure 12. Global temperature estimates at a depth of 10 km [9].
Advanced Geothermal Systems
Advanced geothermal systems (AGS), also known as closed-loop geothermal systems, are designed to work around the challenges faced by traditional and enhanced geothermal systems. Instead of directly extracting geothermal fluids, advanced geothermal systems use closed loops embedded into the ground to transfer heat through a working fluid [26]. By having the loop in direct contact with the reservoir while isolating the working fluid, the issues concerning lack of permeability, thermal short circuits, and working fluid loss are no longer concerns.
Advanced geothermal systems have the potential to be scalable world-wide as they have no dependence on the presence of a reservoir; the only requirement for such systems is to reach temperatures greater than 150-180℃ for electricity generation [4]. Using temperatures as low as 90-120℃ is also possible when coupled with an Organic Rankine Cycle [27, 28]. While AGS sounds like the perfect solution to the issues faced by traditional and enhanced geothermal systems, the technology is still very novel, with little research done on its potential and performance.
One AGS proposed is a closed-loop system in the form of U-shaped wells, comprising two vertical wells, an injection well, and a production well, which are connected laterally (Figure 13) [29]. However, this system comes with efficiency issues as conduction through a pipe is much slower than heat advection [22].
Figure 13. Simple diagram of U-shaped wellbore. [30]
As the years pass, the immediate substrate surrounding the horizontal well becomes cooler due to the conduction rate being faster than the rate of replenishment, and the wells become less efficient. Simulations performed by Song et al. show the impact zone is relatively small, but the temperature decline is large, a 37.49% reduction (Figure 14) [29]. However, the temperature of the fluid, when extracted to depths above 1300 m, remains significantly higher than its surrounding substrate (Figure 14), making the system still potentially viable for energy production [29]. More experimental efforts need to be made to determine the efficiency and viability of implementing U-shaped wells. Advanced geothermal systems are very novel, with relatively low levels of research and production in comparison to traditional and enhanced geothermal systems.
Figure 14. Diagram of simulation displaying impact scope after 20 years [29].
A proposal by Liao et al. suggested the U-shaped well system can be improved by implementing multiple branches (Figure 15) [31]. Numerical simulations show increased power generation and efficiency [31]. Multiple branches increase surface area for conduction while also reducing the flow velocity, which improves thermal recovery by increasing heat exchange time [31].
Figure 15. Diagram of multi-branched U-shaped well system [31].
There is some concern for the impact scope of each horizontal well interfering with one another after drilling, but the numerical simulations from Liao et al. showed that this radius only expands to 20 meters after 20 years (Figure 16) [31]. Similarly, to the results from Song et al., there is a decline in efficiency due to temperature loss, but the recovered temperatures are still relatively high compared to surface substrate temperatures (Figure 17) [29, 31]. After approximately 10 years of exploiting the geothermal resources, the heat exchange will reach a quasi-equilibrium state where it only decreases 0.4℃ per year [31]. If done with proper insulation near boreholes, multi-branch U-shaped wells could be viable for long-term usage.
Figure 16. Changes in horizontal well’s impact zone after 1 month, 1 year, 10 years, and 20 years [31].
Figure 17. Graph displays temperatures of working fluid throughout the system and the changes after 20 years of production [31].
While U-shaped loops are a viable option, they require a great amount of drilling and distance; an alternative option would be to contain the system to vertical wells so former wells from oil and gas mining can be utilized. Green Fire is a company working on GreenLoop, an advanced geothermal system consisting of a tube-in-tube system (Figure 18). The system uses a single vertical well with a smaller, vacuum-insulated liner inside to transport the heated working fluid [32]. The design is intended primarily to make use of the remaining heat from abandoned oil and gas wells. Oil mining utilizes a mature method known as thermal enhanced oil recovery (EOR), which pumps heat in the form of steam to lower the viscosity of oil deposits [32, 33]. When these oil fields are abandoned, years and years of heat remain trapped in the ground, which is what GreenLoop intends to take advantage of. GreenLoop also has the potential to be used in conjunction with active oil fields. Steam can be produced using the extracted heat and reinjected to stimulate more oil production [32].
Figure 18. Diagram of GreenFire Energy’s GreenLoop system [32].
GreenLoop has been tested using working fluids such as water, supercritical CO2, and n-pentane [34]. Water and carbon dioxide are the current ideal choices, as they are environmentally benign if leakages occur [34]. Water was shown to have the highest net energy output in a simulation run by Chandrasekar et al. when coupled with an organic Rankine cycle [35]. Water has traditionally been the working fluid, but supercritical carbon dioxide and n-pentane have also been explored as alternatives.
Several researchers under Green Fire Energy have found that supercritical carbon dioxide was more efficient as it had the ability to flow using a thermosiphon mechanism, eliminating the use of an external pump, but the downside was having lower power outputs [34, 35, 36].
The incentive to research n-pentane despite the risk of environmental pollution is due to its high energy output, like water, while also not requiring a surface heat exchanger [34], due to n-pentane’s lower boiling point. In the past few years, casing manufactures have claimed to produce zero-leakage linings [35], but as closed-loop systems are not well researched, these linings are also yet to be widely tested.
Eavor, a Canadian company specializing in geothermal, is also working on its closed-loop geothermal system called the Eavor-Loop. Construction has started in Germany, with estimates that commercial energy production will start in 2024 and the plant will reach full capacity by 2026 [37]. It uses a radiator-like system using horizontal wells in closed loops 4500 meters below the surface [38, 39]. The system functions similarly to small-scale geothermal heat pumps but does not require an external pump. It takes advantage of thermosiphon movement to transport their proprietary fluid throughout the system [39]. These mechanisms allow the Eavor-Loop to be used for industrial-scale applications. Little is publicly known about the technology behind the Eavor-Loop, but if the project is successful, it could potentially be scalable and adapted easily for any location around the world.
Hybrid Systems and Batteries
Geothermal systems can be used in conjunction with variable sources of energy, such as solar or wind. In addition to using geothermal systems as a storage system, they can also be used as a baseload power source for solar. As geothermal is an invariable power source, it is well suited to being a baseload, but the downside is the inflexibility to increase power output like oil, gas, or solar on occasion. On the other hand, solar power has increased output during warmer seasons and midday, which is useful for providing additional power production during peak hours. Most research on hybrid systems has primarily been done between geothermal and solar, as they complement each other’s weaknesses well. Both can theoretically be implemented everywhere, unlike other renewable sources like wind or hydro. A study conducted by Jiang et al. showed combining geothermal and solar energy systems led to higher efficiency with a 20% power output increase [40].
Geothermal systems are not just for energy production; they also have the potential to be used as batteries during times of increased solar production. The ground can remain at constant temperatures only a few feet below the ground due to low thermal diffusion. In substrates with low permeability, heat is better retained, as seen with enhanced geothermal systems. Like advanced geothermal systems, geothermal batteries make use of existing wells from oil and gas. The wells can be injected with hot fluid containing the excess energy produced from solar or wind power generation (Figure 19) [41]. During simulated sessions of this process, results showcased that 82% of the thermal energy injected can be recovered, and 73% of recovered energy can be successfully used to generate electricity (Figure 20) [41].
Figure 19. Diagram of a geothermal battery [41].
Figure 20. Average efficiencies over 60 cycles (10 years), alternating between withdrawing and injecting heat [42].
Conclusion
Geothermal is a highly promising source of energy to replace fossil fuels while also supplementing existing renewable sources such as solar. However, it is a highly underutilized renewable energy source, mainly due to site dependency and the lack of new technology. While the technology for geothermal heating is mature, the technology for electricity generation is still underdeveloped for commercial use outside of a few select areas.
The main issues faced in expanding geothermal implementation are due to the temperature and depths required for new systems, such as enhanced and advanced geothermal plants. There has been research in plasma and mm-wave drilling to overcome the limitations listed previously, but these have yet to be tested for geothermal sites. In addition, the risk of earthquakes must be considered when developing new drilling methods. In the area of advanced geothermal systems, efforts have been made to develop the most efficiently shaped wells, some being loop-in-loop, U-shaped, or radiator-like. Research into these closed-loop systems have found n-pentane to be the most promising if casing technology evolves to be completely leakproof.
Concurrently, efforts have been made to utilize geothermal sources to supplement the variability of renewable sources such as solar. Depleted geothermal reservoirs can be used to store excess solar energy and be withdrawn during low production times. There is much potential for geothermal systems, but advances in the field are slow compared to other energy fields. If geothermal is to replace oil and gas in addition to being a major renewable energy player in the next 30 years, much more effort needs to be put into developing the necessary drilling and monitoring technologies to expand beyond traditional geothermal systems.
Works Cited
[1] “Fast Facts About Geothermal Energy.” Stanford University Understand Energy Learning
Hub. https://understand-energy.stanford.edu/energy-resources/renewable-energy/geothermal-energy (accessed Jan. 15th, 2024)
[2] “Geothermal Basics.” U.S Office of Energy Efficiency & Renewable Energy.
https://www.energy.gov/eere/geothermal/geothermal-basics (accessed Jan. 14, 2024)
[3] “Geothermal explained.” U.S Energy Information Administration.
https://www.eia.gov/energyexplained/geothermal. (accessed January 14, 2024)
[4] “Global Geothermal Market and Technology Assessment.” International Renewable
Energy Agency (IRENA) and International Geothermal Association (IGA). 2023
[5] L. Kumar, M.S. Hossain, M.H. Assad, M.U. Manoo, “Technological Advancements and
Challenges of Geothermal Energy Systems: A Comprehensive Review.” Energies,
vol. 15, 2022, https://doi.org/10.3390/en15239058
[6] “Geothermal Heat Pumps.” U.S Department of Energy: Energy Saver.
https://www.energy.gov/energysaver/geothermal-heat-pumps (accessed Jan. 16, 2024)
[7] “International Electricity.” U.S. Energy Information Administration.
https://www.eia.gov/international/data/world/electricity/electricity-capacity
[8] A.S. Oyewo, A. Aghahosseini, M.M. Movsessian, C. Breyer, “A novel geothermal-PV led
energy system analysis on the case of the central American countries Guatemala, Honduras, and Costa Rica.” Renewable Energy, vol. 221, article. 119859, 2024, https://doi.org/10.1016/j.renene.2023.119859
[9] A. Aghahosseini, C. Breyer, “From hot rock to useful energy: A global estimate of
enhanced geothermal systems potential.” Applied Energy, vol. 279, article. 115769, 2020, https://doi.org/10.1016/j.apenergy.2020.115769
[10] “Geothermal Energy.” International Renewable Energy Agency (IRENA).
https://www.irena.org/Energy-Transition/Technology/Geothermal-energy (accessed Jan. 15, 2024)
[11] “Electricity Generation.” U.S Office of Energy Efficiency & Renewable Energy.
https://www.energy.gov/eere/geothermal/electricity-generation (accessed Jan. 14, 2024)
[12] S. Earle, “Physical Geology.”
https://opentextbc.ca/geology/chapter/14-1-groundwater-and-aquifers/
[13] R. Moska, K. Labus, P. Kasza, “Hydraulic Fracturing in Enhanced Geothermal
Systems—Field, Tectonic and Rock Mechanics Conditions—A Review” Energies, 2021, https://doi.org/10.3390/en14185725
[14] B. Plumber, “There’s a Vast Source of Clean Energy Beneath Our Feet. And a Race to
Tap It.” The New York Times. https://www.nytimes.com/2023/08/28/climate/geothermal-energy-projects.html (accessed Jan. 16, 2024)
[15] A. De La Garza, “How a Former Oil Guy is Using Fracking Tech to Boost Geothermal
Energy.” Time. https://time.com/6302342/fervo-fracking-technology-geothermal-energy/ (accessed Jan. 16, 2024)
[16] D. Blackwell, M. Richards, Z. Frone, A. Ruzo, R. Dingwall, M. Williams,
“Temperature-At-Depth Maps for the Conterminous US and Geothermal Resource Estimates.” Geothermal Resources Council Transactions, vol. 35, 2011, https://www.smu.edu/dedman/academics/departments/Earth-Sciences/Research/GeothermalLab/DataMaps/TemperatureMaps (accessed Jan. 17, 2024)
[17] “Geothermal Resource Data, Tools, and Maps.” National Renewable Energy Laboratory.
https://www.nrel.gov/gis/geothermal.html
[18] T. Reinsch, P. Dobson, H, Asanuma, E. Huenges, F. Poletto, B. Sanjuan, “Utilizing
supercritical geothermal systems: a review of past ventures and ongoing research activities.” Geothermal Energy 5, article. 16, 2017, https://doi.org/10.1186/s40517-017-0075-y
[19] J. Simpson, “Conventional Petroleum (Oil) and Natural Gas.” Dynamic Planet: Exploring
Geological Disasters and Environmental Change https://open.maricopa.edu/hazards/chapter/10-4-petroleum/
[20] S. Lu, “A global review of enhanced geothermal system (EGS).” Renewable and
Sustainable Energy Reviews, vol. 81, pp. 2902-2921, 2018 http://dx.doi.org/10.1016/j.rser.2017.06.097
[21] “Hydraulic Fracturing.” University of Calgary: Energy Education
https://energyeducation.ca/encyclopedia/Hydraulic_fracturing
[22] A.E. Malek, B.M. Adams, E. Rossi, H.O. Schiegg, “Techno-economic analysis of
Advanced Geothermal Systems (AGS)” Renewable Energy, vol. 186, pp. 927-943, 2022, https://doi.org/10.1016/j.renene.2022.01.012
[23] E.E. Brodsky, L.J. Lajoie, “Anthropogenic Seismicity Rates and Operational Parameters at
the Salton Sea Geothermal Field.” Science, vol. 341, pp. 543-546, 2013, https://www.science.org/doi/full/10.1126/science.1239213
[24] C. Cariaga, “CAFT publishes comprehensive study on superhot rock energy.”
ThinkGeoEnergy, https://www.thinkgeoenergy.com/catf-publishes-comprehensive-study-on-superhot-rock-energy/ (accessed Jan. 31)
[25] A. Albertsson, J.O. Bjarnason, T. Gunnarsson, C. Ballzus, “The Iceland Deep Drilling
Project: Fluid handling, Evaluation and Utilization”, in International Geothermal Conference, Session 6, Sept. 2003, https://www.researchgate.net/publication/268432520
[26] A. Van Horn, A. Amaya, B. Higgins, J. Muir, J. Scherer, R. Pilko, M. Ross, “New
Opportunities and Applications for Closed-Loop Geothermal Energy Systems” Geothermal Resources Council Transactions, vol. 44, 2020, https://www.researchgate.net/publication/358912441_New_Opportunities_and_Applications_for_Closed-Loop_Geothermal_Energy_Systems
[27] “Organic Rankine Cycle” Frigel,
https://www.frigel.com/organic-rankine-cycle-orc-cooling (accessed Feb. 2, 2024)
[28] “Products” Rank, https://www.rank-orc.com/products/ (accessed Feb. 2. 2024)
[29] X. Song, et al. “Numerical analysis of the heat production performance of a closed loop
geothermal system.” Renewable Energy, vol. 120, pp. 365-378, 2018, https://doi.org/10.1016/j.renene.2017.12.065
[30] Y. Ma, S. Li, L. Zhang, S. Liu, M. Wang, “Heat extraction performance evaluation of
U-shaped well geothermal production system under different well-layout parameters and engineering schemes.” Renewable Energy, vol. 203, pp. 473-484, 2023, https://doi.org/10.1016/j.renene.2022.12.082
[31] Y. Liao, X. Sun, B. Sun, Z. Wang, J. Wang, X. Wang, “Geothermal exploitation and
electricity generation from multibranch U-shaped well-enhanced geothermal system.” Renewable Energy, vol. 163, pp. 2178-2189, Jan. 2021, https://doi.org/10.1016/j.renene.2020.10.090
[32] G. Golla, I. Chandra, K. McCarthy, J. Scherer, H. Chin, R. Klenner, “Repurposing oil and
gas wells to produce electricity using the GreenLoop closed-loop geothermal technology.” in Indonesian Petroleum Association, Fourty-Seventh Annual Convention & Exhibition, May 2023, https://www.researchgate.net/publication/374617355_Repurposing_oil_and_gas_wells_to_produce_electricity_using_Closed-Loop_Geothermal_CLG_technology
[33] R. Alvarado, S. Molina, H. Chandrasekar, A. Amaya, “Residual Heat Extraction from
Depleted Heavy-Oil Wells in Thermal EOR.” Geothermal Resources Council Transactions, vol. 47, 2023, https://www.researchgate.net/publication/374471795_Residual_Heat_Extraction_from_Depleted_Heavy-Oil_Wells_in_Thermal_EOR
[34] H. Chandrasekar, A. Amaya, S. Molina, R. Alvarado, “Comparison of Water, sCO2, and
Organic Hydrocarbons as Working Fluids for the GreenLoop System and ORC Unit.” Geothermal Reservoir Engineering, 48th Workshop, Feb. 2023, https://www.researchgate.net/publication/368416783_Comparison_of_Water_sCO2_and_Organic_Hydrocarbons_as_Working_Fluids_for_the_GreenLoop_System_and_ORC_Unit
[35] J. Scherer, G. Golla, H. Chandrasekar, A. Amaya, “The GreenLoop Closed-Loop
Geothermal (CLG) System: Optimizing Power Generation and Evaluating Its Impact on the Reservoir.” Indonesia International Geothermal Convention & Exhibition, Ninth, Sept. 2023, https://www.researchgate.net/publication/374194542_The_GreenLoop_Closed-Loop_Geothermal_CLG_System_Optimizing_Power_Generation_and_Evaluating_Its_Impact_on_the_Reservoir
[36] A. Amaya, J. Scherer, J. Muir, M. Patel, B. Higgins, “GreenFire Energy Closed-Loop
Geothermal Demonstration using Supercritical Carbon Dioxide as Working Fluid.” Geothermal Reservoir Engineering, 45th Workshop, Feb. 2020, https://www.greenfireenergy.com/greenfire-energy-closed-loop-geothermal-demonstration-using-supercritical-carbon-dioxide-as-working-fluid/
[37] “Eavor has broken ground on the first commercial Eavor-Loop project in Germany.”
Eavor. https://www.eavor.com/blog/eavor-has-broken-ground-on-the-first-commercial-eavor-loop-project-in-germany/ (accessed Jan. 15, 2024)
[38] “Closed-Loop Geothermal Technology for a 24/7 Carbon-free and Secure Energy
Future.” Eavor. https://www.eavor.com/technology/ (accessed Jan. 15, 2024)
[39] “Technology and facts.” Eavor. https://eavor-geretsried.de/en/technology-and-facts/
(accessed Jan 15, 2024)
[40] D. Moya, C. Aldás, P. Kaparaju, “Geothermal energy: Power plant technology and direct
heat applications.” Renewable and Sustainable Energy Reviews, vol. 94, pp. 889-901, 2018, https://doi.org/10.1016/j.rser.2018.06.047
[41] J. Jello, M. Khan, N. Malkewicz, S. Whittaker, T. Baser, “Advanced geothermal energy
storage systems by repurposing existing oil and gas wells: A full-scale experimental and numerical investigation.” Renewable Energy, vol. 199, pp. 852-865, Nov. 2022, https://doi.org/10.1016/j.renene.2022.07.145
[42] Q. Zheng, “Design of A High Temperature Subsurface Thermal Energy Storage System.”
MS Thesis, Dept. Hydrogeology, Clemson Univ., Clemson, SC, USA, 2014. https://tigerprints.clemson.edu/all_theses/2008
Dr. Raj Shah Ms. Cindy Huang, Mr.Gavin Thomas Mr.Nikhil Pai Ms.Mrinaleni Das Mr. Nicholas Douglas
Dr. Raj Shah serves in the role of Director at Koehler Instrument Company in New York, boasting an impressive 28-year tenure with the organization. Recognized as a Fellow by eminent organizations such as IChemE, CMI, STLE, AIC, NLGI, INSTMC, Institute of Physics, The Energy Institute, and The Royal Society of Chemistry, he stands as a distinguished recipient of the ASTM Eagle award. Dr. Shah, a luminary in the field, recently coedited the highly acclaimed "Fuels and Lubricants Handbook," a bestseller that unravels industry insights. Explore the intricacies at ASTM's Long-Awaited Fuels and Lubricants Handbook 2nd Edition Now Available (https://bit.ly/3u2e6GY).
His academic journey includes a doctorate in Chemical Engineering from The Pennsylvania State University, complemented by the title of Fellow from The Chartered Management Institute, London. Dr. Shah holds the esteemed status of a Chartered Scientist with the Science Council, a Chartered Petroleum Engineer with the Energy Institute, and a Chartered Engineer with the Engineering Council, UK. Recently honored as "Eminent Engineer" by Tau Beta Pi, the largest engineering society in the USA, Dr. Shah serves on the Advisory Board of Directors at Farmingdale University (Mechanical Technology), Auburn University (Tribology), SUNY Farmingdale (Engineering Management), and the State University of NY, Stony Brook (Chemical Engineering/Material Science and Engineering).
In tandem with his role as an Adjunct Professor at the State University of New York, Stony Brook, in the Department of Material Science and Chemical Engineering, Dr. Shah's impact spans over three decades in the energy industry, with a prolific portfolio of over 625 publications. Dive deeper into Dr. Raj Shah's journey at https://bit.ly/3QvfaLX.
For further correspondence, reach out to Dr. Shah at rshah@koehlerinstrument.com.
Simultaneously, within the dynamic internship program at Koehler Instrument Company in Holtsville, Ms. Cindy Huang , Mr.Gavin Thomas, Mr.Nikhil Pai, Ms.Mrinaleni Das, Mr. Nicholas Douglas are standout participants. They are all students of Engineering at various US universities.
The content & opinions in this article are the author’s and do not necessarily represent the views of AltEnergyMag
Comments (0)
This post does not have any comments. Be the first to leave a comment below.
Featured Product
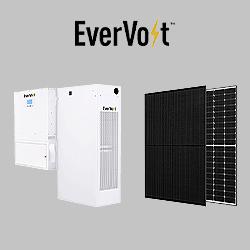